Key Takeaways
1. Structures are everywhere, in nature and technology.
after all, every plant and animal and nearly all of the works of man have to sustain greater or less mechanical forces without breaking, and so practically everything is a structure of one kind or another.
Ubiquitous presence. Structures are not limited to buildings and bridges; they encompass nearly everything that must withstand mechanical forces. From the smallest organism to the largest human creation, the need to support loads without breaking defines a structure. This includes biological forms and technological devices alike.
Nature's engineering. Biological structures existed long before artificial ones, evolving sophisticated solutions for strength and flexibility. Plants and animals, like trees and tendons, demonstrate ingenious ways to manage loads, often surpassing human engineering in efficiency and adaptability. Understanding these natural designs can inform and improve our own technological structures.
Beyond the obvious. The study of structures extends to seemingly simple objects and complex systems. It asks not only why buildings stand but also how biological tissues function, why certain designs are aesthetically pleasing, and how historical artifacts were built without modern theory. This broad perspective reveals the fundamental role of structural principles in our world.
2. Understanding structures requires overcoming communication barriers.
...when they come to tell other people about their subject, something goes badly wrong, for they talk in a strange language...
The Babel problem. Engineers often use specialized language and mathematical concepts that make the study of structures seem incomprehensible to outsiders. This communication gap prevents a wider understanding of a subject deeply relevant to everyday life, biology, and art. Overcoming this 'babble' is essential for broader appreciation and application.
Beyond mathematics. While higher elasticity theory can be complex and mathematical, many fundamental structural concepts are accessible to intelligent laymen. The mystique surrounding the subject is often overdone, hindering practical understanding and application by those in related fields like medicine, biology, and architecture. A simpler, more intuitive approach is needed.
Historical resistance. Historically, non-engineers, including doctors and biologists, have resisted incorporating structural ideas into their fields, partly due to temperament and language barriers. This resistance persists despite the clear relevance of strength, flexibility, and toughness to biological systems and medical practice. Acknowledging the structural element in nature is crucial for progress.
3. All structures resist loads by pushing or pulling back.
Always and whatever happens every force must be balanced and reacted by another equal and opposite force at every point throughout a structure.
Action and reaction. Based on Newton's third law, any force applied to a structure must be met by an equal and opposite force from the structure itself. Whether it's a building pushing down on its foundations or a brick pulling on a string, equilibrium is essential for stability. If forces are unbalanced, the structure will either break or move.
Equilibrium is key. This principle of force balance applies universally, from the simplest string supporting a weight to the most complex bridge or biological system. Every push and pull must be countered at every point within the structure. Failure to achieve this balance results in collapse or unwanted motion.
Passive resistance. Inert materials like stone or steel, or biological tissues like bone or tendon, generate these reactive forces through internal mechanisms. Unlike active muscular reactions, this resistance is passive, arising from the material's inherent properties when deformed. Understanding how this passive resistance is generated is fundamental.
4. Solids resist loads by changing shape – this is elasticity.
Every kind of solid changes its shape – by stretching or contracting itself- when a mechanical force is applied to it.
Hooke's fundamental insight. Robert Hooke realized that solids resist loads by deforming elastically. When a force is applied, the material stretches or compresses, and this change in shape generates the necessary reactive force. This deflection is not a flaw but an essential characteristic of structural behavior.
Deflection is normal. All materials and structures deflect under load, though the extent varies greatly. These movements, even if too small to see, are real and measurable. The science of elasticity studies the relationship between these forces and deflections.
- Cathedral columns bend under weight.
- Strings lengthen when pulled.
- Bridges sag under traffic.
Internal resistance. Hooke correctly inferred that this macroscopic change in shape is due to the deformation of internal bonds between atoms and molecules. These strong bonds resist being stretched or compressed, generating the large forces needed to balance external loads.
5. Stress and Strain are fundamental concepts for quantifying material behavior.
The concept of the elastic conditions at a specified point inside a material is the concept of stress and strain.
Quantifying internal state. Stress is a measure of the internal force per unit area within a material, akin to pressure in a fluid but often directional. Strain is the corresponding measure of deformation, representing the proportionate change in length or shape. These concepts allow engineers to analyze the conditions at any point within a loaded solid.
Stress vs. Strain. Stress (force/area) tells us how hard atoms are being pushed or pulled; strain (change in length/original length) tells us how far they are being displaced. They are distinct but related.
- Stress units: p.s.i., MN/m², kgf/cm²
- Strain units: dimensionless ratio (often expressed as percentage)
Young's Modulus. For many materials, stress is proportional to strain within the elastic limit (Hooke's Law). The ratio of stress to strain is Young's Modulus (E), a measure of the material's stiffness. A high E means the material is stiff; a low E means it is flexible.
6. Strength and Stiffness are distinct material properties.
A biscuit is stiff but weak, steel is stiff and strong, nylon is flexible (low E) and strong, raspberry jelly is flexible (low E) and weak.
Defining properties. Strength refers to the stress a material can withstand before breaking (e.g., tensile strength). Stiffness, quantified by Young's Modulus (E), describes how much a material deforms under a given stress. These properties are independent and crucial for material selection.
Examples illustrate difference:
- Biscuit: High stiffness (doesn't bend easily), low strength (breaks easily).
- Steel: High stiffness, high strength.
- Nylon: Low stiffness (flexible), high strength.
- Jelly: Low stiffness, low strength.
Structural implications. A structure needs to be both strong enough not to break and stiff enough not to deflect excessively for its intended purpose. A wobbly table or a sagging floor, even if strong enough not to collapse, is unacceptable. Design often involves balancing these two requirements.
7. Geometric irregularities cause dangerous stress concentrations.
Geometrical irregularities, such as holes and cracks and sharp corners, which had previously been ignored, may raise the local stress – often only over a very small area – very dramatically indeed.
Hidden dangers. Stress concentrations occur at points where the shape of a structure changes abruptly, like around holes, notches, or cracks. These irregularities force stress lines to crowd together, causing the local stress to be much higher than the average stress in the material. This was a key insight from C. E. Inglis.
Magnified stress. The local stress at the tip of a sharp crack can be hundreds or even thousands of times higher than the nominal stress elsewhere. This explains why structures can fail at loads far below what simple calculations predict, as seen in ship failures like the Majestic or Leviathan.
- Grooves in chocolate bars facilitate breaking.
- Nicks in fabric selvedges help tearing.
- Sharp corners in hatchways caused ship cracks.
Adding material can weaken. Counterintuitively, adding stiff patches or extra material can also cause stress concentrations if they disrupt the smooth flow of stress, potentially weakening the structure. This highlights the complexity of load distribution.
8. Fracture is an energy-driven process, not just about stress.
Modern fracture mechanics is therefore less concerned with forces and stresses than with how, why, where and when strain energy can be turned into fracture energy.
Energy balance. A. A. Griffith's theory showed that fracture is fundamentally an energy conversion process. A crack will propagate only if the release of stored elastic strain energy is sufficient to pay the energy cost of creating new fracture surfaces (the 'work of fracture'). This explains why structures with high local stress don't always break.
Critical crack length. For any given material and stress level, there is a critical crack length (Lg). Cracks shorter than Lg are stable and won't propagate spontaneously; cracks longer than Lg are unstable and will spread rapidly, leading to catastrophic failure. This provides a defense against stress concentrations.
- Lg depends on work of fracture (W), Young's Modulus (E), and stress (s).
- Lg = 2WE/s² (simplified).
- Higher W and E, lower s lead to larger Lg (safer).
Toughness matters. The 'work of fracture' or 'toughness' of a material (energy needed to create new surface) is crucial. Brittle materials like glass have low work of fracture, making them susceptible to crack propagation even at low stress. Tough materials like steel have high work of fracture, requiring much more energy to break.
9. Materials fail differently in tension, compression, and shear.
What is really happening in failures of this sort is that the material or the structure finds some way of evading an unduly high compressive stress, usually by moving ‘out from under’ the load...
Evasion in compression. Unlike tension, where failure involves pulling bonds apart, compression failure often involves the material or structure shifting sideways to escape the load. This can happen through various mechanisms depending on the material and geometry.
Failure modes:
- Short, brittle struts: Fail by shearing along planes at 45° to the load (e.g., concrete crushing).
- Short, ductile struts: Bulge outwards due to plastic flow by shear (e.g., metal upsetting).
- Fibrous materials: Form compression creases or buckles in fibers (e.g., wood).
- Long, thin members: Buckle sideways elastically (Euler buckling).
Strength variation. The strength of a material can be vastly different in tension and compression. Cast iron is strong in compression but weak in tension, while wood is stronger in tension than compression. This asymmetry must be considered in design, leading to non-symmetrical beam cross-sections.
10. Joints are critical points where structures often fail.
...when a shore carpenter is building a house or putting together traditional furniture he is in the habit of making joints which a naval architect or an engineer would regard as weak and highly inefficient.
Load transfer challenge. Joints connect structural elements and must efficiently transfer loads between them. This process often creates stress concentrations, making joints inherently weaker than the continuous material. The design and execution of joints are paramount for structural integrity.
Efficiency vs. reliability. Traditional joints (e.g., pegged timber, riveted plates) might seem 'inefficient' to modern engineers focused on minimal material use, but they often offer valuable flexibility or redundancy. This 'give' can help redistribute loads and prevent catastrophic failure, unlike rigid, 'efficient' joints that may fail suddenly.
- Riveted joints can slip slightly, relieving stress concentrations.
- Wooden joints allow for timber expansion/contraction.
- Glued joints, while strong, can fail catastrophically if imperfect.
Human factor. The reliability of joints, especially those requiring manual skill like welding or gluing, is highly dependent on human conscientiousness. Accidents often stem from poorly made joints, highlighting the gap between theoretical design and practical execution. Inspection is difficult, making trust and skill vital.
11. Long, thin members fail by buckling, not crushing.
A long rod, or a membrane such as a thin sheet of metal or a page of this book, fails in compression by buckling, as can very easily be seen by doing the simplest experiment.
Euler's insight. Leonhard Euler analyzed the failure of long, thin columns under compression, showing they fail by bending sideways (buckling) rather than crushing. The buckling load depends on the material's stiffness (E), the cross-section's shape (I), and the length (L), but not the material's strength.
Buckling load formula: P = π²EI/L² (for pin-jointed ends). This shows that increasing length dramatically reduces the load a column can support before buckling. A long strut is much weaker in compression than a short one of the same material and cross-section.
Design implications. To prevent buckling in long compression members, they must be made significantly thicker or shaped to increase their 'second moment of area' (I). Tubes are efficient shapes for resisting buckling. Thin panels and shells also buckle, requiring stiffening elements like ribs, stringers, or sandwich construction to increase their stability.
12. Design is a compromise balancing competing structural requirements.
In this respect most structures have to be a compromise between stiffness and strength and resilience, and the achievement of the best compromise is likely to tax the skill of a designer severely.
Balancing virtues. Structural design is rarely about maximizing a single property but about finding the optimal balance between conflicting requirements. A structure needs sufficient strength, stiffness, resilience (energy absorption), and often minimum weight and cost. These goals are frequently incompatible.
Examples of compromise:
- Stiffness vs. Resilience: A very stiff structure may be strong but brittle under impact; a resilient one absorbs energy but may be too flexible for its purpose (e.g., car suspension).
- Strength vs. Toughness: High tensile strength often comes with low toughness, making materials susceptible to crack propagation (e.g., high tensile steel vs. mild steel).
- Weight vs. Strength/Stiffness: Reducing weight often requires using materials or designs that are more complex or expensive, or accepting lower safety margins (e.g., aircraft vs. buildings).
Context matters. The 'best' design and material choice depend entirely on the specific application and its priorities. What works for a building (stability, low stress) is different from what works for an aircraft (low weight, high strength/stiffness). Nature's designs, evolved through competition, represent highly optimized compromises for specific ecological niches.
Last updated:
Review Summary
Structures receives mostly positive reviews for its accessible explanation of engineering concepts, engaging writing style, and broad scope covering buildings, bridges, and biological structures. Readers appreciate Gordon's humor, historical anecdotes, and ability to convey complex ideas to laypeople. Some criticize the book's organization and occasional digressions. Many find it eye-opening, providing new perspectives on the built environment. The final chapter on aesthetics surprises some readers. Overall, it's considered a valuable introduction to structural engineering for non-specialists.
Similar Books
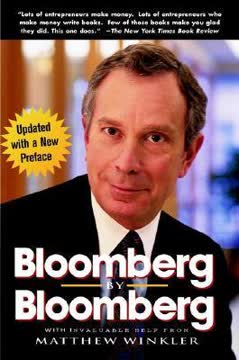
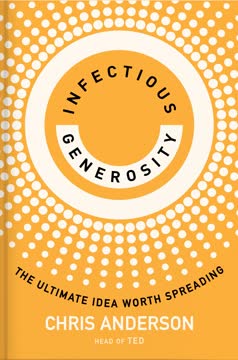
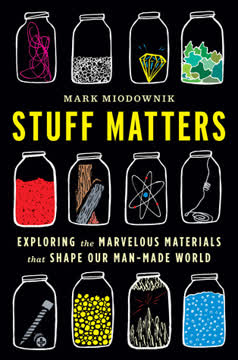
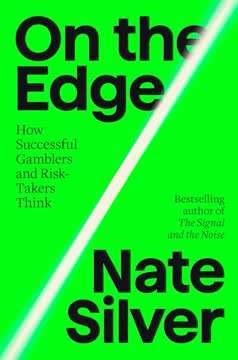
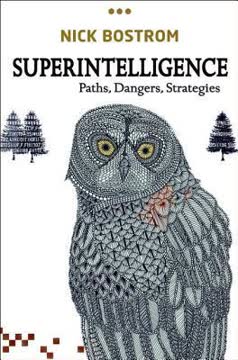
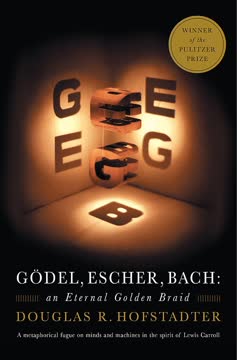
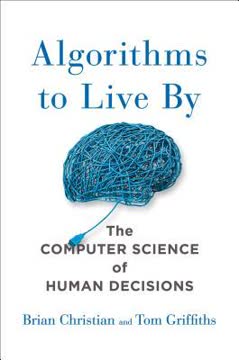
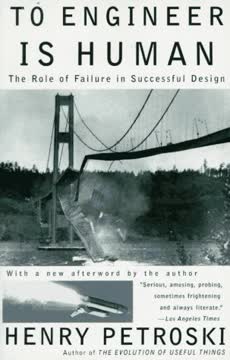
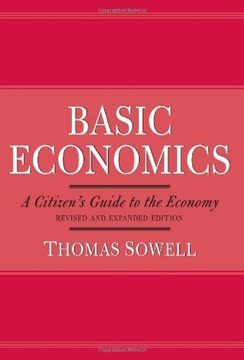
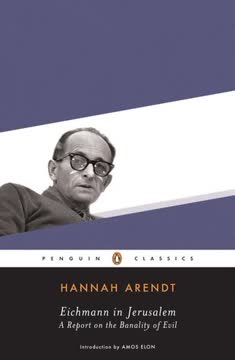
Download PDF
Download EPUB
.epub
digital book format is ideal for reading ebooks on phones, tablets, and e-readers.